How is a long strand of human genome organized in the cell??
Research Summary
Since Flemming described a nuclear substance in the 19th century and named
it “chromatin”, this substance has fascinated biologists. What is the structure
of chromatin? Human genome DNA is wrapped around core histones, forming
a nucleosome fiber (10-nm fiber). This fiber has long been assumed to fold
into a 30-nm chromatin fiber, and subsequently into helically folded larger
fibers, or radial loops. However, our cryo-EM (1, 2) and X-ray scattering
analyses (3,4) demonstrated that chromatin is composed of irregularly folded
10-nm fibers, without 30-nm chromatin fibers, in interphase chromatin and
mitotic chromosomes. This irregular folding implies a chromatin state that
is physically less constrained, which could be more dynamic compared with
classical regular helical folding structures. Consistent with this, recently
using single nucleosome imaging, we uncovered by single nucleosome imaging
large nucleosome fluctuations in living mammalian cells (~50 nm/30 ms)
(5,6). Subsequent computational modeling suggested that nucleosome fluctuation
increases chromatin accessibility, which is advantageous for many “target
searching” biological processes such as transcriptional regulation. Therefore,
we have provided a novel view on chromatin structure, in which chromatin
consists of dynamic and disordered 10-nm fibers (polymer-melt)(2,7).
1. Eltsov et al. (2008) Proc Natl Acad Sci U S A 105, 19732-19737.
2. Maeshima et al. (2010) Curr Opin Cell Biol 22, 291-297.
3. Nishino et al. (2012) EMBO J. 31, 1644-53.
4. Joti et al. (2012) Nucleus 3, 404-410.
5. Hihara et al. (2012) Cell Reports 2, 1645-56.
6. Nozaki et al. (2013) Nucleus 4, 3349-356.
7. Maeshima et al. (2014) Chromosoma 123, 225-237
More detailed description
Introductory movie by Virginia Hughes
The following research description was modified from Maeshima et al., 2014.
For more comprehensive description in the chromatin field, see Maeshima et al., 2016.
Background
There are 60 trillion cells in the human body. Each cell contains 2 m
of genomic DNA in a small nucleus with an approximately 10-mm diameter
(a volume of only ~100 fL to 1 pL), and yet it is able to search and read
the information in its genomic DNA to execute diverse cellular functions.
Therefore, it is important to understand how this long genomic DNA is organized
in the nucleus. In the 19th century, W. Flemming described a nuclear substance
that was clearly visible after staining with a basic dye using primitive
light microscopes, and named it “chromatin.” This is now thought to be
the basic unit of genomic DNA organization (Olins and Olins 2003). Since
then, even before the discovery of the structure of DNA (Watson and Crick
1953), chromatin has attracted significant interest from biologists.
DNA and nucleosomes
Deoxyribonucleic acid (DNA) is a negatively charged polymer that produces
electrostatic repulsion between adjacent DNA regions. Therefore, it would
be difficult for a long DNA molecule alone to fold into a small space like
the nucleus (Bloomfield 1996). To overcome this problem, the long, negatively
charged polymer is wrapped around a basic protein complex known as a core
histone octamer, which consists of the histone proteins H2A, H2B, H3, and
H4, to form a nucleosome (Fig. 1)(Olins and Olins 1974; Kornberg 1974;
Woodcock et al. 1976). This nucleosome fiber is also known as the 10-nm
fiber (Fig. 1). A single histone octamer in the nucleosome has ~220 positively
charged lysine and arginine residues, and ~74 negatively charged aspartic
acid and glutamic acid residues. There are also 400 negative charges in
the phosphate backbone of 200 bp of DNA. Because only about half of the
negative charges in the DNA are neutralized, the remaining charge must
be neutralized by other factors (e.g., linker histone H1, cations, and
other positively charged molecules) for further folding.
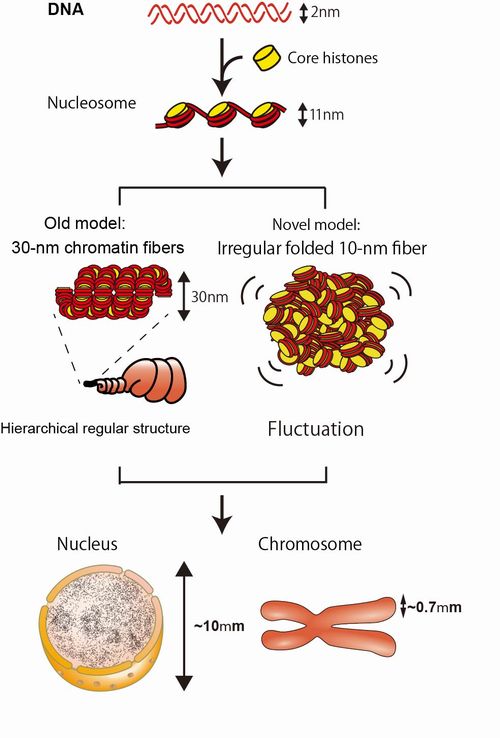
Figure 1.Old and novel views of chromatin structure.
A long DNA molecule with a diameter of ~2 nm is wrapped around a core
histone octamer, and forms a nucleosome with a diameter of 11 nm. The nucleosome
has long been assumed to fold into 30-nm chromatin fibers (left), and subsequently
into the higher-order organization of interphase nuclei or mitotic chromosomes.
The right panel shows the novel hypothesis of irregularly folded nucleosome
fibers.
30-nm chromatin fibers and further helical holding structures
In 1976, Finch and Klug first found, under transmission electron microscopy
(EM), that purified nucleosome fibers (10-nm fibers) with linker histone
H1 or Mg2+ ions were folded into fibers with a diameter of 30 nm. They
named these fibers ‘30-nm chromatin fibers’ (Figs. 1, 2A, and 2B) (Finch
and Klug 1976). In their structural model of the 30-nm fibers called “solenoids”,
consecutive nucleosomes are located adjacent to one another in the fiber,
and fold into a simple “one-start helix” (Fig. 2A). Subsequently, a second
model of the “two-start helix” was proposed based on microscopic observations
of isolated nucleosomes (Fig. 2B) (Woodcock et al. 1984). The second model
assumed that nucleosomes were arranged in a zigzag manner, where a nucleosome
in the fiber was bound to the second neighbor (Bassett et al. 2009) (Fig.
2B). In addition to these two famous structural models, many other structural
variations of 30-nm chromatin fibers have been proposed (van Holde and
Zlatanova 2007).
It has long been assumed that the 10-nm nucleosome fibers form a 30-nm chromatin fiber, and subsequently the higher-order chromatin structures of interphase nuclei and mitotic chromosomes. Several models have been proposed to describe the structure of higher-order chromatin. The “hierarchical helical folding model” suggests that a 30-nm chromatin fiber is folded progressively into larger fibers, including ~100-nm and then ~200-nm fibers, to form large interphase chromatin fibers (chromonema fibers) or mitotic chromosomes (Fig. 2C) (Sedat and Manuelidis 1978; Belmont et al. 1989; Belmont and Bruce 1994). In contrast, the “radial loop model” assumes that a 30-nm chromatin fiber folds into radially oriented loops to form mitotic chromosomes (Fig. 2C) (Paulson and Laemmli 1977; Laemmli et al. 1978; Marsden and Laemmli 1979).
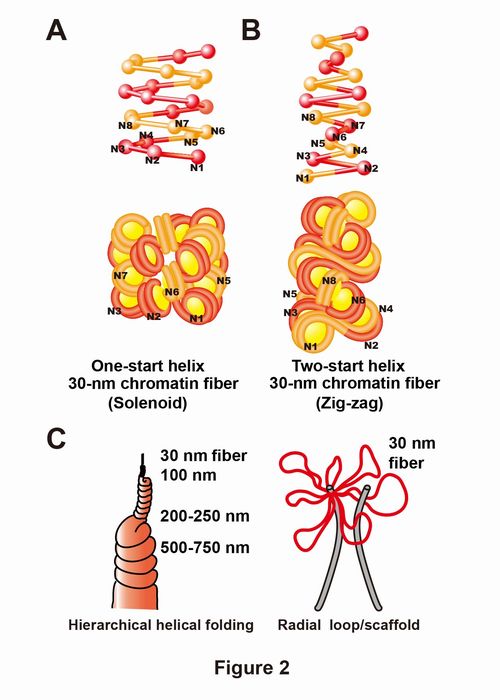
Figure 2.Two classical models of 30-nm chromatin fibers and higher-order chromatin
structures.
(A) One-start helix (solenoid); (B) two-start helix (zigzag). (Top) A scheme
of the two different topologies of chromatin fibers is shown Robinson and
Rhodes (2006). Positions from the first (N1) to the eighth (N8) nucleosome
are labeled. (C) Two classical higher-order chromatin structure models:
the radial loop model (Laemmli et al., 1978), and the hierarchical helical
folding model (Sedat and Manuelidis, 1978). In the radial loop model, many
loop structures of the 30-nm fiber (red) wrap around the scaffold structure
(gray) (Laemmli et al., 1978), which consists of condensin and topoisomerase
IIα (Maeshima and Laemmli, 2003).
Does the 30-nm chromatin fiber exist in vivo? The cryo-electron microscopy
(EM) study
In 1986, the Dubochet group performed a pioneering study to visualize
native cellular structures using cryo-EM (McDowall et al. 1986). Mammalian
mitotic cells were frozen rapidly, sectioned, and observed directly under
a cryo-EM with no chemical fixation or staining (cryo-EM of vitreous sections;
CEMOVIS). Surprisingly, the chromosomes had a homogeneous, grainy texture
with ~11-nm spacing. No higher-order or periodic structures, including
30-nm fibers, were observed.
Interphase chromatin has also been visualized using cryo-EM. It was suggested
that interphase nuclei in most higher eukaryote cells might not contain
30-nm chromatin fibers (Dubochet et al. 2001; Bouchet-Marquis et al. 2006;
Fakan and van Driel 2007).
On the other hand, it is unclear whether the absence of 30-nm structures
in cryo-EM images truly demonstrates a lack of 30-nm chromatin fibers because
when researchers capture cryo-EM images they use a technique called “defocusing”
to produce high-contrast images. This process results in artificial amplification
or suppression of the signal intensity, which affects different structural
features depending on the defocus value (contrast transfer function [CTF]
effect; for a review, see Frank 2006). It is thus possible that the degree
of defocusing needed to image chromosomes or chromatin with high contrast
prevents the visualization of 30-nm chromatin fibers.
To solve this problem, our laboratory collaborated with Eltsov, Frangakis,
and Dubochet to compensate for the CTF effect by merging several images
taken at different levels of defocus into a single image (Conway and Steven
1999). Even after this correction, we were unable to detect 30-nm structures
in the chromosomal areas. In addition, the detection of periodic structures
in the chromosomal region by power spectral (Fourier transform) analysis
revealed a prominent peak at 11 nm, but not at 30 nm. This cryo-EM study
suggested that 30-nm chromatin fibers were essentially absent from mitotic
chromosomes; therefore, we proposed that 10-nm nucleosome fibers exist
in a highly disordered, interdigitated state similar to a “polymer melt”
(Figs. 1 and 4) (Eltsov et al. 2008; Maeshima et al. 2010).
Small angle X-ray scattering analyses revealed no 30-nm chromatin structures
in interphase nuclei and mitotic chromosomes
Although our cryo-EM study did not detect any 30-nm structures in mitotic
chromosomes, it might have been challenging to observe potential hierarchical
regular structures because only a small number of 50-nm sections were examined
(Eltsov et al. 2008). Langmore and Paulson (Langmore and Paulson 1983;
Paulson and Langmore 1983) detected a 30-nm structure in interphase nuclei
and mitotic chromosomes using small angle X-ray scattering (SAXS) analysis,
which can detect bulky periodic structures in non-crystal materials in
solution without chemical fixation or staining (Fig. 3A and B). Therefore,
this study provided evidence for the existence of 30-nm chromatin fibers
in interphase chromatin and mitotic chromosomes (Langmore and Paulson 1983;
Paulson and Langmore 1983). Because these findings were inconsistent with
the cryo-EM findings described above, we performed a comprehensive investigation
of the structure of interphase nuclei and mitotic chromosomes using SAXS
and cryo-EM (Nishino et al. 2012; Joti et al. 2012; for review, see Hansen
2012). Isolated human interphase nuclei and mitotic chromosomes were exposed
to synchrotron X-ray beams (Fig. 3A). A typical scattering pattern of interphase
nuclei and mitotic chromosomes exhibited three peaks at 30-, weakly at
11-, and 6-nm (Fig. 3C, left) (Nishino et al. 2012; Joti et al. 2012).
This was consistent with the previous findings of Langmore and Paulson
(1983), who suggested that the 6- and 11-nm peaks were derived from the
face-to-face and edge-to-edge positioning of nucleosomes, respectively.
They concluded that the 30-nm peak represented the side-by-side packaging
of 30-nm chromatin fibers. However, this fails to explain why the 30-nm
structures were not observed in interphase chromatin and mitotic chromosomes
using cryo-EM.
To understand the nature of the 30-nm peak observed using SAXS, isolated chromosomes were examined using cryo-EM (Nishino et al. 2012; Joti et al. 2012). Again, no 30-nm chromatin fibers were observed in chromosomes. However, the cryo-EM images revealed that the surface of the chromosome was coated with electron-dense granules the size of ribosomes. Subsequent immunostaining and western blotting confirmed that the chromosome surface was contaminated with ribosomes. The ribosomes were stacked regularly at ~30-nm intervals, which could explain the ~30-nm peak observed using SAXS. To test this hypothesis, we removed ribosomes from the surface of the chromosome by washing with an isotonic buffer containing polyamine and EDTA while maintaining the size and shape of the chromosomes, and then analyzed mitotic chromosomes using SAXS. Importantly, no 30-nm peaks were detected (Fig 3C, right), but the 11- and 6-nm peaks resulting from the internal structure of the nucleosomes remained (Fig. 3C, right). Similarly, when we examined nuclei after ribosome removal, the 30-nm peak in the SAXS pattern disappeared (Joti et al. 2012). These results suggested the absence of a 30-nm chromatin fiber in interphase chromatin and mitotic chromosomes.
Next, we investigated the larger-scale chromatin structure of interphase nuclei and mitotic chromosomes using a newly developed apparatus for ultra-small-angle X-ray scattering (USAXS) (Nishino et al. 2009). Consistent with our previous observations, there were no regular periodic structures, between ~30- and 1000- nm in interphase nuclei and mitotic chromosomes. This contradicts the hierarchical helical folding model (Figure 2C)(Nishino et al. 2012; Joti et al. 2012). The scattering properties also suggested the existence of a scale-free structure or fractal nature up to ~275-nm in interphase chromatin, and ~1000-nm in mitotic chromosomes. This suggests that interphase and mitotic chromatin share the common structural features of up to ~275 nm of condensed and irregularly folded 10-nm nucleosome fibers, without 30-nm structures (discussed below). Taken together, the cryo-EM, SAXS, and USAXS data suggest that irregularly folded 10-nm nucleosome fibers form the bulk structure of human interphase chromatin and mitotic chromosomes (Nishino et al. 2012; Joti et al. 2012).
Other evidence supporting the absence of 30-nm chromatin fibers
Dekker (2008) used the chromosome-conformation-capture (3C) technique to
investigate the folding of a specific genomic DNA region within yeast cells.
He measured the average distance between two loci in the genome by confocal
microscopy and the flexibility of the intervening chromatin fiber by the
3C technique. In combination with polymer modeling, the mass density of
the chromatin fiber was determined. His conclusion was that yeast chromatin
in a transcriptionally active domain did not form a compact 30-nm chromatin
fiber but, rather, was extended with a loose arrangement of 10-nm nucleosome
fibers.
More recently, Bazett-Jones et al. (2008) used electron spectroscopic imaging (ESI), a process that involves electron microscopy with an energy filter. ESI makes it possible to perform phosphorus and nitrogen mapping in cells with high contrast and resolution (Ahmed et al. 2009; Fussner et al. 2011). The signals from phosphorus and nitrogen, which are the main components of DNA, may be used to assess the folding of genomic DNA, and can distinguish 10- from 30-nm fibers. They observed that pluripotent cells were characterized by a highly dispersed mesh of 10-nm, but not 30-nm, fibers (Fussner et al. 2011; Fussner et al. 2012). In contrast, differentiated cells form compact chromatin domains that leave a large space in the nucleus that is devoid of DNA. Surprisingly, ESI combined with tomography methods revealed that condensed heterochromatin domains such as chromocenters consisted of 10-nm, rather than 30-nm, chromatin fibers (Fussner et al. 2012), consistent with the observations using cryo-EM. Furthermore, Gan et al. investigated the picoplankton Ostreococcus tauri, the smallest known free-living eukaryote, using cryo-EM tomography of ice sections and subsequent computational analysis (Gan et al. 2013). They demonstrated that O. tauri chromatin resembles a disordered assembly of nucleosomes without the 30-nm chromatin structure, compatible with the polymer melt model. Therefore, several lines of evidence suggest the absence of regular 30-nm chromatin fibers in eukaryotic cells.
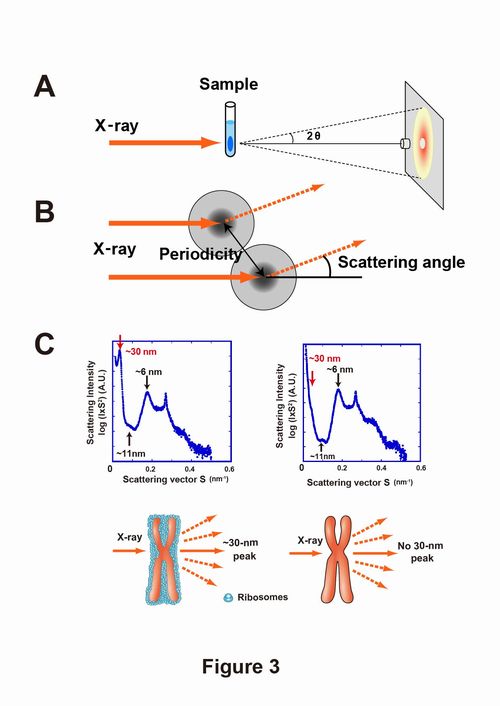
Figure 3.Small angle X-ray scattering (SAXS) analysis of chromatin structure. (A) Experimental design. The chromosome pellet in a quartz capillary tube was exposed to synchrotron X-ray beams, and the scattering patterns were recorded using the imaging plate (Nishino et al., 2012). (B) When non-crystal materials were irradiated with X-rays, scattering at small-angles generally reflected periodic structures. Images (A) and (B) were reproduced from (Joti et al., 2012), with some modifications. (C) (Upper left) Typical SAXS patterns of purified mitotic HeLa chromosome fractions. Three peaks at ~6, ~11 (weak), and ~30 nm were detected (arrows). (Upper right) After the removal of ribosome aggregates, the 30-nm peak disappeared, whereas the other peaks remained. (Bottom) A model whereby the 30-nm peak in SAXS results from regularly spaced ribosome aggregates, and not from the chromosomes. Image (C) was reproduced from (Nishino et al., 2012), with some modification.
Although the near absence of 30-nm chromatin fibers in eukaryotic cells was suggested, these structures are shown in EM images in molecular biology textbooks. We propose that most 30 nm chromatin fibers in EM images are in vitro artifacts caused by the low salt buffer conditions. The formation of 30-nm chromatin fibers requires the selective binding of nucleosomes, which are close neighbors on the DNA strand, via intra-fiber nucleosomal association (Fig. 4A). In low salt buffer conditions of <1 mM MgCl2 or <100 mM NaCl, nucleosomal fibers gently repel each other due to their negative charges. This “isolation of nucleosome fibers” facilitates the intra-fiber nucleosomal association and the subsequent formation of stable 30-nm chromatin fibers (Figs. 4A and B). In conventional EM imaging studies, these 30-nm fibers might be stabilized through chemical cross-linking (such as glutaraldehyde fixation) and then shrunk further after alcohol dehydration during sample preparation (Maeshima et al. 2010).
Polymer melt
It is important to assess chromatin structure under more physiological
salt conditions. Under these conditions, inter-fiber nucleosome interactions
become increasingly dominant (Fig. 4A and B) (Maeshima et al. 2010). Nucleosome
fibers (10 nm) are forced to interdigitate, which interferes with the formation
and maintenance of 30-nm chromatin fibers. This leads to the “polymer melt”
(Maeshima et al. 2010) or “self-oligomer” state (for review, see Hansen
2002; Hansen 2012)) (Fig. 4A and B). In addition, inter-fiber nucleosome
interactions increase significantly in the presence of >2 mM Mg2+
ions (Zheng et al. 2005; Kan et al. 2009). However, it is important to
note that the tail domain of histone H4 mediates both 30 nm fiber formation
(Dorigo et al. 2003) and inter-fiber nucleosome association (Kan et al.
2009). Consequently, inter-fiber nucleosome association can prevent the
formation of 30-nm fibers by sequestering the H4 tail domain (Hansen 2012).
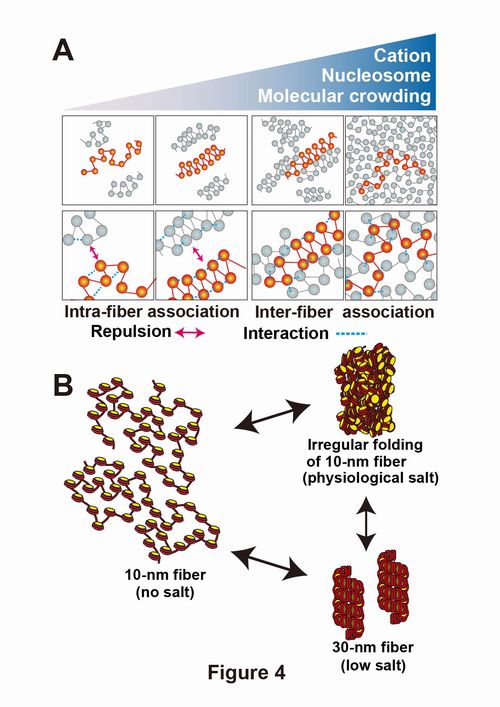
Figure 4.Polymer-melt model.
(A) Under low-salt conditions, nucleosome fibers could form 30-nm chromatin
fibers via intra-fiber nucleosome associations. An increase in salt (cation)
concentration results in inter-fiber nucleosomal contacts that interfere
with intra-fiber nucleosomal associations, leading to a polymer melt scenario.
Note that in these illustrations, we show a highly simplified two-dimensional
nucleosome model. Arrows and dotted lines show repulsion forces and interactions,
respectively. (B) During the melting process, the 30-nm chromatin fibers
become irregularly folded nucleosome fibers.
Dynamic 10-nm fibers in living mammalian cells
The original liquid chromatin model proposed by Dubochet (McDowall et al.,
1986; Dubochet et al. 1988) and our polymer melt model (Eltsov et al.,
2008; Maeshima et al., 2010) both imply a less physically constrained chromatin
state, and a more dynamic state locally; the 10-nm nucleosome fibers fluctuate
locally. Therefore, we attempted to visualize local nucleosome fluctuation.
Previous studies of chromatin dynamics employed very large chromatin regions
such as the LacO array that encompasses 20-50 nucleosomes (Straight et
al., 1996; Belmont et l., 1999; Heun et al., 2001; Vazquezet al., 2001;
Chubb et al., 2002; Levi et al., 2005; Hajjoul et al., 2013). The motion
of these large regions in living mammalian cells was measured by monitoring
the movement of the GFP-LacI signal bound to the LacO array at specific
chromatin regions.
To observe and analyze more local nucleosome dynamics, we performed single nucleosome imaging in living mammalian cells (Fig. 5) (Hihara et al., 2012; Nozaki et al., 2013). We fused histone H4 with photoactivatable (PA)-GFP, and expressed the fusion protein in mammalian cells at a very low level (Fig. 5A). We then used an oblique illumination microscope to illuminate a limited thin area within the cell for single nucleosome imaging (Hihara et al., 2012; Nozaki et al., 2013; for principle, see Tokunaga et al., 2008). Generally, PA-GFP shows green fluorescence only after activation with a 405-nm laser (Lippincott-Schwartz et al., 2009). Surprisingly, we observed that a small fraction of H4-PA-GFP and PA-GFP-H4 in the cells was activated spontaneously without laser stimulation (Fig. 5A). Fig. 5B shows a typical single nucleosome image of a living mammalian cell. Each bright dot in the nucleus represents a single H4-PA-GFP (PA-GFP-H4) within the single nucleosome. Strikingly, we observed significant nucleosome fluctuation (~50 nm movement/30 ms) in both interphase chromatin and mitotic chromosomes (Fig. 5C), likely caused by Brownian motion (Hihara et al., 2012; Nozaki et al., 2013). Mean square displacement (MSD) plots, measuring the spatial extent of the random motion, and fitting to an anomalous diffusion curve suggested a restricted nucleosome movement. The McNally group also published single nucleosome tracking data using H2B-EGFP (Mazza et al., 2012), which appear to be consistent with our single nucleosome tracking results using PA-GFP-H4.
Local fluctuation of nucleosomes as a basis for scanning genome information
Some computational modeling studies, including our own, have suggested
that nucleosome fluctuations facilitate the mobility of diffusing proteins
in the chromatin environment (Fig. 5D) (Wedemeier et al., 2009; Fritsch
and Langowski 2011; Hihara et al., 2012). Such nucleosome fluctuations
may also contribute to the frequent exposure of genomic DNA sequences.
Because both facilitating protein mobility and DNA exposure increase chromatin
accessibility, these local dynamics may be advantageous in template-directed
biological processes such as transcriptional regulation, DNA replication,
and DNA repair/recombination. Therefore, we propose that the local fluctuation
of nucleosomes forms the basis for scanning genome information (Fig. 5D).
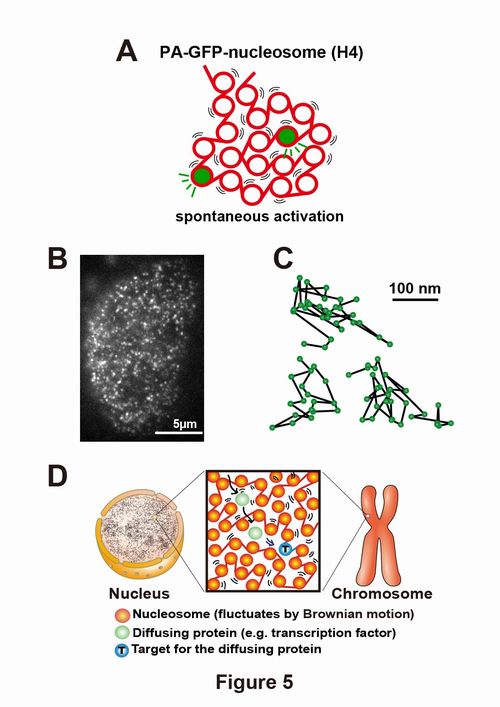
Figure 5. Single nucleosome imaging.
(A) A small portion of PA-GFP-H4 was activated spontaneously without laser
activation, and was used for single nucleosome imaging. (B) Single nucleosome
image of a DM cell (Indian Muntjac cell) nucleus that expresses PA-GFP-H4.
PA-GFP-H4 is observed as a bright dot using oblique illumination microscopy.
The dots were fitted to an assumed Gaussian point spread function to determine
the precise center of signals with higher resolution. Bar = 5 µm. (C) Representative
three trajectories of fluorescently tagged single nucleosomes. (D) Chromatin
fluctuations as a basis for scanning genome information. In cells, nucleosome
fibers (red spheres and lines) are folded irregularly. The nucleosomes
fluctuate, and these nucleosome dynamics facilitate chromatin accessibility.
The images were reproduced from (Hihara et al. 2012; Nozaki et al., 2013)
Conclusions
The traditional view of chromatin is changing from one of static regular
structures including 30-nm chromatin fibers to a dynamic irregular folding
structure of 10-nm nucleosome fibers. Although the term “irregular” or
“disordered” might give the impression that the organization is functionally
irrelevant, the irregular folding results in less physical constraint and
increased dynamism, increasing the accessibility of the DNA (Fig. 5D).
This dynamic state may be essential for various genome functions, including
transcription, replication, and DNA repair/recombination.
References
Ahmed K, Li R, Bazett-Jones DP (2009) Electron spectroscopic imaging of
the nuclear landscape. Methods Mol Biol 464: 415-423.
Bassett A, Cooper S, Wu C, Travers A (2009) The folding and unfolding of eukaryotic chromatin. Curr Opin Genet Dev 19: 159-165.
Bazett-Jones DP, Li R, Fussner E, Nisman R, Dehghani H (2008) Elucidating chromatin and nuclear domain architecture with electron spectroscopic imaging. Chromosome Res 16: 397-412.
Belmont AS, Braunfeld MB, Sedat JW, Agard DA (1989) Large-scale chromatin structural domains within mitotic and interphase chromosomes in vivo and in vitro. Chromosoma 98: 129-143.
Belmont AS, Bruce K (1994) Visualization of G1 chromosomes: a folded, twisted, supercoiled chromonema model of interphase chromatid structure. J Cell Biol 127: 287-302.
Belmont AS, Li G, Sudlow G, Robinett C (1999) Visualization of large-scale chromatin structure and dynamics using the lac operator/lac repressor reporter system. Methods Cell Biol 58: 203-222.
Bloomfield VA (1996) DNA condensation. Curr Opin Struct Biol 6: 334-341.
Bordas J, Perez-Grau L, Koch MH, Vega MC, Nave C (1986) The superstructure of chromatin and its condensation mechanism. I. Synchrotron radiation X-ray scattering results. Eur Biophys J 13: 157-173.
Bouchet-Marquis C, Dubochet J, Fakan S (2006) Cryoelectron microscopy of vitrified sections: a new challenge for the analysis of functional nuclear architecture. Histochem Cell Biol 125: 43-51.
Chubb JR, Boyle S, Perry P, Bickmore WA (2002) Chromatin motion is constrained by association with nuclear compartments in human cells. Curr Biol 12: 439-445.
Conway JF, Steven AC (1999) Methods for reconstructing density maps of "single" particles from cryoelectron micrographs to subnanometer resolution. J Struct Biol 128: 106-118.
Dekker J (2008) Mapping in vivo chromatin interactions in yeast suggests an extended chromatin fiber with regional variation in compaction. J Biol Chem 283: 34532-34540.
Dorigo B, Schalch T, Bystricky K, Richmond TJ (2003) Chromatin fiber folding: requirement for the histone H4 N-terminal tail. J Mol Biol 327: 85-96.
Dubochet J, Adrian M, Chang JJ, Homo JC, Lepault J, McDowall AW, Schultz P (1988) Cryo-electron microscopy of vitrified specimens. Q Rev Biophys 21: 129-228.
Dubochet J, Sartori Blanc N (2001) The cell in absence of aggregation artifacts. Micron 32: 91-99.
Eltsov M, Maclellan KM, Maeshima K, Frangakis AS, Dubochet J (2008) Analysis of cryo-electron microscopy images does not support the existence of 30-nm chromatin fibers in mitotic chromosomes in situ. Proc Natl Acad Sci U S A 105: 19732-19737.
Fakan S, van Driel R (2007) The perichromatin region: a functional compartment in the nucleus that determines large-scale chromatin folding. Semin Cell Dev Biol 18: 676-681.
Finch JT, Klug A (1976) Solenoidal model for superstructure in chromatin. Proc Natl Acad Sci U S A 73: 1897-1901.
Frank J (2006) Three-Dimensional Electron Microscopy of Macromolecular Assembly.
Fritsch CC, Langowski J (2011) Chromosome dynamics, molecular crowding, and diffusion in the interphase cell nucleus: a Monte Carlo lattice simulation study. Chromosome Res 19: 63-81.
Fussner E, Ching RW, Bazett-Jones DP (2011) Living without 30nm chromatin fibers. Trends Biochem Sci 36: 1-6.
Fussner E, Djuric U, Strauss M, Hotta A, Perez-Iratxeta C, Lanner F, Dilworth FJ, Ellis J, Bazett-Jones DP (2011) Constitutive heterochromatin reorganization during somatic cell reprogramming. Embo J 30: 1778-1789.
Fussner E, Strauss M, Djuric U, Li R, Ahmed K, Hart M, Ellis J, Bazett-Jones DP (2012) Open and closed domains in the mouse genome are configured as 10-nm chromatin fibres. EMBO Rep 13: 992-996.
Gan L, Ladinsky MS, Jensen GJ (2013) Chromatin in a marine picoeukaryote is a disordered assemblage of nucleosomes. Chromosoma 122: 377-386.
Hajjoul H, Mathon J, Ranchon H, Goiffon I, Mozziconacci J, Albert B, Carrivain P, Victor JM, Gadal O, Bystricky K, Bancaud A (2013) High-throughput chromatin motion tracking in living yeast reveals the flexibility of the fiber throughout the genome. Genome Res 23: 1829-1838.
Hansen JC (2002) Conformational dynamics of the chromatin fiber in solution: determinants, mechanisms, and functions. Annu Rev Biophys Biomol Struct 31: 361-392.
Hansen JC (2012) Human mitotic chromosome structure: what happened to the 30-nm fibre? Embo J 31: 1621-1623.
Heun P, Laroche T, Shimada K, Furrer P, Gasser SM (2001) Chromosome dynamics in the yeast interphase nucleus. Science 294: 2181-2186.
Hihara S, Pack CG, Kaizu K, Tani T, Hanafusa T, Nozaki T, Takemoto S, Yoshimi T, Yokota H, Imamoto N, Sako Y, Kinjo M, Takahashi K, Nagai T, Maeshima K (2012) Local nucleosome dynamics facilitate chromatin accessibility in living mammalian cells. Cell Rep 2: 1645-1656.
Joti Y, Hikima T, Nishino Y, Kamada F, Hihara S, Takata H, Ishikawa T, Maeshima K (2012) Chromosomes without a 30-nm chromatin fiber. Nucleus 3: 404-410.
Kan PY, Caterino TL, Hayes JJ (2009) The H4 tail domain participates in intra- and internucleosome interactions with protein and DNA during folding and oligomerization of nucleosome arrays. Mol Cell Biol 29: 538-546.
Kornberg RD (1974) Chromatin structure: a repeating unit of histones and DNA. Science 184: 868-871.
Laemmli UK, Cheng SM, Adolph KW, Paulson JR, Brown JA, Baumbach WR (1978) Metaphase chromosome structure: the role of nonhistone proteins. Cold Spring Harb Symp Quant Biol 42: 351-360.
Langmore JP, Paulson JR (1983) Low angle x-ray diffraction studies of chromatin structure in vivo and in isolated nuclei and metaphase chromosomes. J Cell Biol 96: 1120-1131.
Levi V, Ruan Q, Plutz M, Belmont AS, Gratton E (2005) Chromatin dynamics in interphase cells revealed by tracking in a two-photon excitation microscope. Biophys J 89: 4275-4285.
Lippincott-Schwartz J, Patterson GH (2009) Photoactivatable fluorescent proteins for diffraction-limited and super-resolution imaging. Trends Cell Biol 19: 555-565.
Maeshima K, Eltsov M (2008) Packaging the genome: the structure of mitotic chromosomes. J Biochem (Tokyo) 143: 145-153.
Maeshima K, Hihara S, Eltsov M (2010) Chromatin structure: does the 30-nm fibre exist in vivo? Curr Opin Cell Biol 22: 291-297.
Maeshima, K.,Imai, R.,Tamura, S., Nozaki,T. (2014) Chromatin as dynamic 10-nm fibers. Chromosoma 123: 225-237.
Maeshima K, Laemmli UK (2003) A two-step scaffolding model for mitotic chromosome assembly. Dev Cell 4: 467-480.
Marsden MP, Laemmli UK (1979) Metaphase chromosome structure: evidence for a radial loop model. Cell 17: 849-858.
Mazza D, Abernathy A, Golob N, Morisaki T, McNally JG (2012) A benchmark for chromatin binding measurements in live cells. Nucleic Acids Res 40: e119.
McDowall AW, Smith JM, Dubochet J (1986) Cryo-electron microscopy of vitrified chromosomes in situ. Embo J 5: 1395-1402.
Nishino Y, Eltsov M, Joti Y, Ito K, Takata H, Takahashi Y, Hihara S, Frangakis AS, Imamoto N, Ishikawa T, Maeshima K (2012) Human mitotic chromosomes consist predominantly of irregularly folded nucleosome fibres without a 30-nm chromatin structure. Embo J 31: 1644-1653.
Nishino Y, Takahashi Y, Imamoto N, Ishikawa T, Maeshima K (2009) Three-Dimensional Visualization of a Human Chromosome Using Coherent X-ray Diffraction. . Physical Review Letters 102: 18101 (18104 pages)
Nozaki T, Kaizu K, Pack CG, Tamura S, Tani T, Hihara S, Nagai T, Takahashi K, Maeshima K (2013) Flexible and dynamic nucleosome fiber in living mammalian cells. Nucleus 4: 349-356.
Olins AL, Olins DE (1974) Spheroid chromatin units (v bodies). Science 183: 330-332.
Olins DE, Olins AL (2003) Chromatin history: our view from the bridge. Nat Rev Mol Cell Biol 4: 809-814.
Paulson JR, Laemmli UK (1977) The structure of histone-depleted metaphase chromosomes. Cell 12: 817-828.
Paulson JR, Langmore JP (1983) Low angle x-ray diffraction studies of HeLa metaphase chromosomes: effects of histone phosphorylation and chromosome isolation procedure. J Cell Biol 96: 1132-1137.
Robinson PJ, Rhodes D (2006) Structure of the '30 nm' chromatin fibre: a key role for the linker histone. Curr Opin Struct Biol 16: 336-343.
Sedat J, Manuelidis L (1978) A direct approach to the structure of eukaryotic chromosomes. Cold Spring Harb Symp Quant Biol 42 Pt 1: 331-350.
Straight AF, Belmont AS, Robinett CC, Murray AW (1996) GFP tagging of budding yeast chromosomes reveals that protein-protein interactions can mediate sister chromatid cohesion. Curr Biol 6: 1599-1608.
Tokunaga M, Imamoto N, Sakata-Sogawa K (2008) Highly inclined thin illumination enables clear single-molecule imaging in cells. Nat Methods 5: 159-161.
van Holde K, Zlatanova J (2007) Chromatin fiber structure: Where is the problem now? Semin Cell Dev Biol 18: 651-658.
Vazquez J, Belmont AS, Sedat JW (2001) Multiple regimes of constrained chromosome motion are regulated in the interphase Drosophila nucleus. Curr Biol 11: 1227-1239.
Watson JD, Crick FH (1953) Molecular structure of nucleic acids; a structure for deoxyribose nucleic acid. Nature 171: 737-738.
Wedemeier A, Merlitz H, Wu CX, Langowski J (2009) How proteins squeeze through polymer networks: a Cartesian lattice study. J Chem Phys 131: 064905 (064907 pages).
Woodcock CL, Frado LL, Rattner JB (1984) The higher-order structure of chromatin: evidence for a helical ribbon arrangement. J Cell Biol 99: 42-52.
Woodcock CL, Safer JP, Stanchfield JE (1976) Structural repeating units in chromatin. I. Evidence for their general occurrence. Exp Cell Res 97: 101-110.
Zheng C, Lu X, Hansen JC, Hayes JJ (2005) Salt-dependent intra- and internucleosomal
interactions of the H3 tail domain in a model oligonucleosomal array. J
Biol Chem 280: 33552-33557.
▲Page top